Ybco (isbn 979-8340057846)

Table of contents
- Chapter 1: The Quest for Higher Temperature Superconductors
- Chapter 2: The Breakthrough: The Discovery of YBCO
- Chapter 3: The Race to Understand YBCO
- Chapter 4: The Synthesis and Characterization of YBCO
- Chapter 5: The Crystal Structure of YBCO
- Chapter 6: The Electronic Properties of YBCO
- Chapter 7: The Magnetic Properties of YBCO
- Chapter 8: The Optical Properties of YBCO
- Chapter 9: YBCO in Power Transmission
- Chapter 10: YBCO in Magnetic Levitation
- Chapter 11: YBCO in Electronics
- Chapter 12: YBCO in Medicine
- Chapter 13: The Search for Even Higher Tc Superconductors
- Chapter 14: The Challenges of Commercializing YBCO
- Chapter 15: The Impact of YBCO on Society and Technology
- Chapter 16: The Environmental Impact of YBCO
- Chapter 17: The Role of YBCO in Scientific Research
- Chapter 18: The Role of Doping in YBCO
- Chapter 19: The Anisotropy of YBCO
- Chapter 20: The Theory of High-Temperature Superconductivity
- Chapter 21: The Future of YBCO and Superconductivity
- Chapter 22: Levitation Technology
- Chapter 23: Aviation and YBCO
- Chapter 24: Floating and YBCO
- Chapter 25: Materials Similar to YBCO
- Chapter 26: The Quest for Higher Temperature Superconductors
- Chapter 27: Superconductivity in Extreme Conditions
- Chapter 28: Superconducting Quantum Computing
- Chapter 29: Energy Storage and YBCO
- Chapter 30: The Future of YBCO and Superconductivity
- Chapter 31: Ethical Considerations and Sustainability
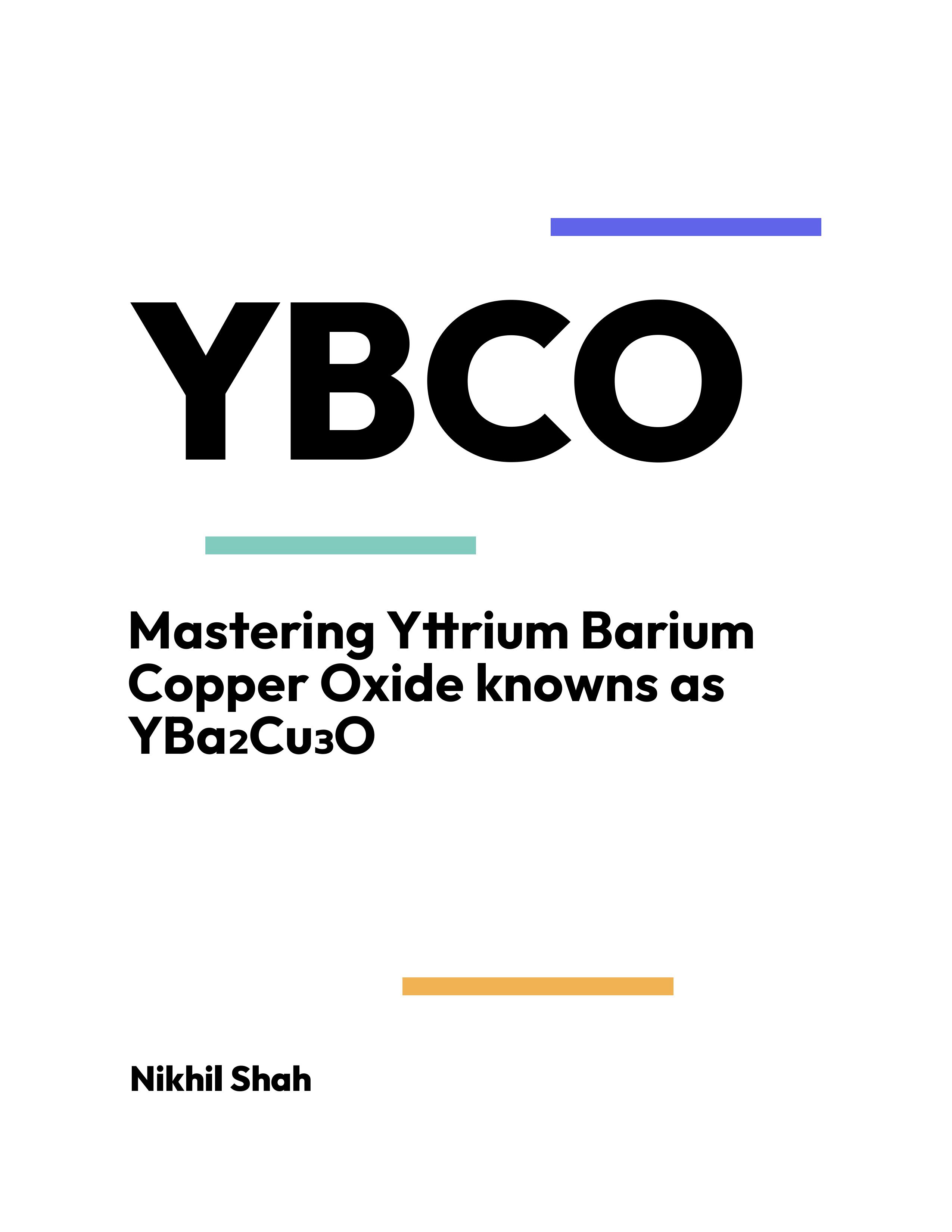
Chapter 1: The Quest for Higher Temperature Superconductors
The Dawn of a New Era
The discovery of superconductivity, a phenomenon where certain materials exhibit zero electrical resistance below a critical temperature, marked a turning point in the history of physics. This groundbreaking discovery, made independently by Dutch physicists Heike Kamerlingh Onnes and Walther Meissner in the early 20th century, opened up new possibilities for technological advancements. However, the low critical temperatures of early superconductors, typically below 20 Kelvin (-253 degrees Celsius), severely limited their practical applications.
The Limitations of Low-Temperature Superconductors
Low-temperature superconductors, such as lead and tin, required expensive and complex cryogenic systems to maintain their superconducting state. These systems were bulky, energy-intensive, and often impractical for large-scale applications. As a result, the potential of superconductivity remained largely untapped for decades.
The Search for Higher Temperature Superconductors
Scientists and engineers alike recognized the immense potential of superconductors with higher critical temperatures. Such materials could enable a wide range of applications, from energy-efficient power transmission to high-speed magnetic levitation (maglev) trains. The quest for higher temperature superconductors became a major research focus in the latter half of the 20th century.
Early Efforts and Challenges
Despite significant research efforts, progress in developing higher temperature superconductors was slow. Researchers explored various materials and techniques, but the elusive goal of a room-temperature superconductor remained out of reach. The challenges were numerous, including the complexity of the materials involved and the difficulty of synthesizing them in pure and reproducible forms.
The Significance of Higher Temperature Superconductors
The potential benefits of higher temperature superconductors are substantial. They could revolutionize energy transmission, transportation, electronics, and medical technology. Some of the key advantages include:
Energy efficiency: Superconducting power transmission lines can transmit electricity with minimal energy loss, reducing the need for costly power plants.
Transportation: Maglev trains powered by superconducting magnets offer the potential for high-speed, energy-efficient transportation.
Electronics: Superconducting devices can operate at much higher speeds and with lower energy consumption than conventional electronic components.
Medical applications: Superconducting magnets are used in magnetic resonance imaging (MRI) machines, providing high-resolution medical imaging.
The discovery of a higher temperature superconductor would have a profound impact on society and technology, opening up new avenues of innovation and improving our quality of life.
Chapter 2: The Breakthrough: The Discovery of YBCO
An Unexpected Discovery
In 1986, a breakthrough in the field of superconductivity occurred that would forever change the landscape of materials science and technology. Karl Alex Müller and Johannes Georg Bednorz, working at IBM's Zurich Research Laboratory, made a serendipitous discovery that would lead to the development of high-temperature superconductors.
Müller and Bednorz were investigating the properties of lanthanum barium copper oxide (LBCO). While their initial focus was on understanding the electronic structure of this material, they stumbled upon something extraordinary. They observed that LBCO exhibited superconductivity at a relatively high temperature of 35 Kelvin (-238 degrees Celsius). This was a significant improvement over the previously known record of 23 Kelvin.
The Significance of the Discovery
The discovery of LBCO was a major milestone in the quest for higher temperature superconductors. It demonstrated that it was possible to achieve superconductivity at temperatures well above the boiling point of liquid nitrogen, a relatively inexpensive and readily available coolant. This opened up new possibilities for practical applications of superconductivity that had previously been limited by the need for expensive cryogenic systems.
The Race to Raise the Critical Temperature
News of the LBCO discovery quickly spread throughout the scientific community, sparking a worldwide race to find materials with even higher critical temperatures. Researchers around the globe began experimenting with different combinations of elements and synthesis techniques, hoping to push the boundaries of superconductivity further.
The Emergence of YBCO
Within a year of the LBCO discovery, a new material emerged as a leading contender for the title of the highest temperature superconductor: yttrium barium copper oxide, or YBCO. Researchers found that by replacing lanthanum with yttrium in the LBCO structure, they could significantly increase the critical temperature. In 1987, a team at the University of Houston reported the synthesis of YBCO with a critical temperature of 93 Kelvin (-180 degrees Celsius). This was a groundbreaking achievement, as it marked the first time a superconductor had been discovered that could operate at temperatures above the boiling point of liquid nitrogen.
The Impact of YBCO
The discovery of YBCO had a profound impact on the field of superconductivity. It not only raised the critical temperature to a more practical level but also spurred further research into the underlying mechanisms of high-temperature superconductivity. YBCO became the benchmark for future developments in the field, and it remains one of the most widely studied and applied high-temperature superconductors today.
Chapter 3: The Race to Understand YBCO
A Global Scientific Frenzy
The discovery of YBCO ignited a global scientific frenzy as researchers raced to understand its properties and explore its potential applications. Laboratories around the world, driven by both scientific curiosity and the promise of technological breakthroughs, poured resources into studying this remarkable new material.
The Challenges of Studying YBCO
Despite the excitement surrounding YBCO, its study presented significant challenges. The complex crystal structure of YBCO, with its intricate arrangement of atoms, made it difficult to synthesize in a pure and consistent form. Moreover, the superconducting properties of YBCO were highly sensitive to factors such as oxygen content, impurities, and processing conditions.
Worldwide Research Efforts
Researchers from diverse backgrounds, including chemists, physicists, materials scientists, and engineers, contributed to the growing body of knowledge about YBCO. They employed a wide range of experimental techniques to probe its properties, including X-ray diffraction, neutron scattering, electron microscopy, and spectroscopy.
Key Areas of Investigation
Crystal structure: Determining the precise arrangement of atoms in the YBCO lattice was crucial for understanding its superconducting properties.
Oxygen content: The oxygen content of YBCO was found to be a critical factor in determining its superconducting transition temperature.
Electronic structure: Researchers sought to understand the electronic states of YBCO and how they contribute to superconductivity.
Magnetic properties: The magnetic behavior of YBCO, including the Meissner effect and flux pinning, was a subject of intense study.
Synthesis and processing: Developing reliable methods for synthesizing and processing YBCO was essential for practical applications.
The Quest for a Unified Theory
One of the most pressing questions facing researchers was the nature of the pairing mechanism responsible for superconductivity in YBCO. Unlike conventional superconductors, where electron pairs are mediated by electron-phonon interactions, the pairing mechanism in high-temperature superconductors remained a mystery. Several competing theories emerged, including those involving magnetic fluctuations and electronic correlations.
The Road Ahead
Despite the challenges, the research community made significant progress in understanding YBCO and other high-temperature superconductors. The insights gained from these studies laid the foundation for future developments in the field and paved the way for potential applications that were once thought to be unattainable.
Chapter 4: The Synthesis and Characterization of YBCO
Creating the Superconductor
The synthesis of YBCO, a complex oxide compound, requires careful control of the chemical composition, temperature, and atmosphere. Researchers have developed various methods to produce high-quality YBCO samples.
Solid-state reaction: This is the most common method, involving mixing the appropriate amounts of yttrium oxide, barium carbonate, and copper oxide powders. The mixture is heated in a furnace under controlled conditions to promote chemical reactions and form YBCO.
Sol-gel method: In this method, the starting materials are dissolved in a liquid to form a sol, which is then converted into a gel. The gel is dried and calcined to produce YBCO powder.
Flux growth: This technique involves melting a mixture of YBCO and a flux material, such as potassium carbonate or sodium carbonate. As the mixture cools, YBCO crystals grow from the flux.
Characterization Techniques
Once YBCO is synthesized, it is essential to characterize its properties to ensure its quality and suitability for specific applications. Various techniques are used to analyze the composition, structure, and superconducting behavior of YBCO.
X-ray diffraction (XRD): This technique determines the crystal structure and lattice parameters of YBCO.
Scanning electron microscopy (SEM): SEM provides high-resolution images of the microstructure of YBCO, revealing its grain size and morphology.
Transmission electron microscopy (TEM): TEM offers even higher resolution and can be used to study the atomic-scale structure of YBCO.
Energy-dispersive X-ray spectroscopy (EDX): EDX can be used to analyze the elemental composition of YBCO.
Magnetic susceptibility measurements: These measurements determine the superconducting transition temperature and the Meissner effect.
Electrical resistivity measurements: These measurements assess the electrical conductivity of YBCO above and below the superconducting transition temperature.
Optimization and Reproducibility
The synthesis and characterization of YBCO require careful optimization to achieve the desired properties. Factors such as the purity of the starting materials, the heating conditions, and the cooling rate can significantly influence the quality of the final product. Ensuring reproducibility is also crucial for reliable and consistent performance in applications.
By mastering the synthesis and characterization of YBCO, researchers have been able to produce high-quality samples suitable for a wide range of applications, from power transmission to electronic devices.
Chapter 5: The Crystal Structure of YBCO
The Building Blocks of Superconductivity
The crystal structure of a material plays a crucial role in determining its physical properties. In the case of YBCO, its unique crystal structure is intimately linked to its ability to exhibit superconductivity.
The Perovskite Structure
YBCO belongs to the perovskite family of crystal structures. Perovskites are characterized by a simple cubic lattice with a large cation (in this case, yttrium) at the center, surrounded by six smaller anions (oxygen) at the corners of the cube.
The Role of Oxygen
The oxygen content of YBCO is a critical factor in determining its superconducting properties. The optimal oxygen content for superconductivity is typically around 6.95 atoms per formula unit. Deviations from this ideal oxygen content can significantly reduce or even eliminate superconductivity.
The Cu-O Planes
Within the YBCO crystal structure, copper atoms are arranged in square planar coordination with oxygen atoms. These Cu-O planes are believed to be the primary conduits for electrical current in the superconducting state. The strong electronic interactions between copper and oxygen atoms in these planes are thought to be essential for the pairing mechanism that gives rise to superconductivity.
The Importance of the Crystal Structure
The crystal structure of YBCO provides a framework for understanding its electronic, magnetic, and superconducting properties. By studying the arrangement of atoms and the interactions between them, researchers can gain insights into the fundamental mechanisms underlying high-temperature superconductivity.
Conclusion
The crystal structure of YBCO, with its perovskite-based architecture and the crucial role of oxygen, is a key factor in its ability to exhibit superconductivity. Understanding the structural details of YBCO provides a foundation for further research into the properties and applications of this remarkable material.
Chapter 6: The Electronic Properties of YBCO
Superconductivity in YBCO
One of the most remarkable properties of YBCO is its ability to exhibit superconductivity at relatively high temperatures. This phenomenon is characterized by the complete absence of electrical resistance below a critical temperature (Tc). In the case of YBCO, Tc can reach as high as 93 Kelvin (-180 degrees Celsius).
The Pairing Mechanism
The mechanism responsible for superconductivity in YBCO remains a subject of intense research. Unlike conventional superconductors, where electron pairs are mediated by electron-phonon interactions, the pairing mechanism in high-temperature superconductors is believed to be more complex.
Several competing theories have been proposed, including:
Magnetic fluctuation theory: This theory suggests that magnetic fluctuations in the Cu-O planes play a crucial role in pairing electrons.
Electronic correlation theory: This theory emphasizes the strong electronic interactions between copper and oxygen atoms in the Cu-O planes.
Excitonic mechanism: This theory proposes that excitons, bound electron-hole pairs, mediate the pairing of electrons.
The Cooper Pair
Regardless of the specific pairing mechanism, superconductivity in YBCO is believed to involve the formation of Cooper pairs. These are pairs of electrons that are bound together by an attractive force. The formation of Cooper pairs allows electrons to move through the material without encountering resistance.
The Energy Gap
In the superconducting state, a region of energy known as the superconducting gap opens up around the Fermi level. This energy gap prevents electrons from scattering and contributes to the zero resistance of the material.
The Role of Doping
The superconducting properties of YBCO can be influenced by doping with other elements, such as rare earth metals or strontium. Doping can alter the electronic structure of YBCO and affect its critical temperature.
Conclusion
The electronic properties of YBCO are complex and fascinating. Understanding the pairing mechanism and the underlying electronic structure of YBCO is essential for developing a comprehensive theory of high-temperature superconductivity and for exploring potential applications of this remarkable material.
Chapter 7: The Magnetic Properties of YBCO
The Meissner Effect
One of the most distinctive properties of superconductors is the Meissner effect. When a superconducting material is cooled below its critical temperature and placed in a magnetic field, it expels the magnetic flux from its interior. This phenomenon is a direct consequence of the pairing of electrons in the superconducting state.
Flux Pinning
While the Meissner effect prevents magnetic fields from penetrating the bulk of a superconductor, it does not completely shield the material from external magnetic fields. Magnetic flux can still enter the superconductor in the form of quantized vortices. These vortices are regions of normal conducting material that allow magnetic flux to penetrate the superconductor.
Flux pinning is the process of trapping these vortices within the superconductor, preventing them from moving freely. This is essential for practical applications of superconductors, as it helps to stabilize the superconducting state and prevent energy losses.
Vortex Dynamics
The behavior of vortices in a superconductor is influenced by factors such as the strength of the applied magnetic field, the temperature, and the presence of defects or impurities in the material. The dynamics of vortices can affect the critical current density and the stability of the superconducting state.
Applications of Flux Pinning
Flux pinning is crucial for applications that involve high magnetic fields, such as superconducting magnets for magnetic resonance imaging (MRI) and particle accelerators. By carefully controlling the density and distribution of pinning centers, it is possible to optimize the performance of these devices.
Conclusion
The magnetic properties of YBCO, including the Meissner effect and flux pinning, are essential for its practical applications. Understanding the behavior of magnetic flux in YBCO is crucial for designing and optimizing superconducting devices.
Chapter 8: The Optical Properties of YBCO
Infrared and Raman Spectroscopy
Infrared and Raman spectroscopy are powerful techniques for studying the vibrational properties of materials. These methods can provide insights into the interactions between atoms and molecules in a material.
In the case of YBCO, infrared and Raman spectroscopy have been used to investigate the vibrational modes of the copper-oxygen lattice. By analyzing the frequencies and intensities of these vibrational modes, researchers can gain information about the electronic structure and the superconducting pairing mechanism.
Optical Conductivity
The optical conductivity of a material measures its ability to absorb and transmit light. In YBCO, the optical conductivity exhibits a significant change across the superconducting transition temperature. Above the critical temperature, YBCO behaves as a normal metal, with a relatively high optical conductivity. Below the critical temperature, however, the optical conductivity drops dramatically due to the formation of the superconducting gap.
The Superconducting Gap
The superconducting gap is a region of energy that separates the occupied and unoccupied electronic states in a superconductor. By studying the optical conductivity of YBCO, researchers can determine the size of the superconducting gap and gain insights into the pairing mechanism.
Conclusion
Infrared and Raman spectroscopy, along with measurements of optical conductivity, provide valuable information about the electronic properties of YBCO. These techniques have played a crucial role in understanding the mechanisms underlying high-temperature superconductivity.
Chapter 9: YBCO in Power Transmission
A Revolution in Energy Efficiency
One of the most promising applications of YBCO is in power transmission. Superconducting cables made from YBCO can transmit electricity with significantly lower energy losses compared to conventional copper or aluminum cables. This is due to the zero electrical resistance of YBCO in its superconducting state.
Potential Benefits
Reduced energy losses: Superconducting cables can reduce energy losses during transmission, leading to significant cost savings.
Increased transmission capacity: Superconducting cables can carry higher currents than conventional cables, allowing for increased transmission capacity.
Improved grid stability: Superconducting cables can help to stabilize the electrical grid by reducing transmission losses and improving fault current capabilities.
Technological Challenges
Despite the potential benefits, there are several technological challenges to overcome before YBCO can be widely adopted for power transmission:
Cooling requirements: YBCO must be cooled to below its critical temperature to maintain its superconducting state. This requires the use of cryogenic systems, which can be expensive and complex.
Manufacturing costs: The manufacturing of superconducting cables is currently more expensive than conventional cables. However, as production volumes increase and manufacturing processes improve, costs are expected to decrease.
Reliability and safety: Ensuring the reliability and safety of superconducting power systems is a critical concern. This requires careful design, testing, and maintenance.
Recent Developments
In recent years, significant progress has been made in addressing these challenges. Researchers and engineers have developed innovative cooling technologies and manufacturing techniques to improve the feasibility of YBCO-based power transmission systems. Additionally, efforts are underway to improve the reliability and safety of these systems.
Conclusion
YBCO has the potential to revolutionize power transmission by enabling more efficient and reliable delivery of electricity. While there are still challenges to overcome, the benefits of superconducting power transmission are substantial and could have a significant impact on the global energy landscape.
Chapter 10: YBCO in Magnetic Levitation
Maglev: A Revolutionary Transportation Technology
Magnetic levitation (maglev) technology offers the potential for high-speed, energy-efficient transportation. By suspending vehicles above tracks using magnetic forces, maglev systems can reduce friction and improve efficiency. YBCO, with its high critical temperature, is a promising material for use in maglev systems.
Maglev Trains
Maglev trains are the most well-known application of maglev technology. These trains use powerful magnets to levitate above a track, reducing friction and allowing for higher speeds. YBCO-based magnets can generate the strong magnetic fields required for maglev trains, making them a potential alternative to conventional magnets.
Benefits of Maglev
High speeds: Maglev trains can travel at much higher speeds than traditional rail systems, reducing travel times.
Energy efficiency: Due to the reduced friction, maglev trains are more energy-efficient than conventional trains.
Reduced noise and vibration: Maglev trains produce less noise and vibration than traditional trains, improving the passenger experience.
Technological Challenges
Despite the promise of maglev technology, there are still several challenges to overcome. These include:
Cost: The initial investment required to build maglev systems is high.
Infrastructure: Building maglev tracks and stations requires significant infrastructure development.
Public acceptance: There may be concerns about the safety and environmental impact of maglev systems.
Recent Developments
In recent years, there has been significant progress in the development of maglev technology. Several countries have built or are planning to build maglev systems, demonstrating the feasibility of this technology.
Conclusion
YBCO-based magnets offer the potential to revolutionize transportation through maglev technology. While there are still challenges to overcome, the benefits of maglev trains in terms of speed, efficiency, and environmental impact make it a promising area for future development.
Chapter 11: YBCO in Electronics
Superconducting Electronic Devices
The unique properties of YBCO offer the potential for the development of novel electronic devices. The zero electrical resistance of YBCO can lead to significant improvements in performance and efficiency.
Josephson Junctions
Josephson junctions are devices made from two superconducting materials separated by a thin insulating barrier. These devices exhibit a variety of quantum phenomena, including the Josephson effect, which allows for the direct conversion of electrical signals into microwave radiation.
YBCO-based Josephson junctions have been used in a variety of electronic applications, including:
Microwave mixers: These devices are used to combine signals from different frequencies.
SQUIDs (Superconducting Quantum Interference Devices): SQUIDs are extremely sensitive magnetometers that can detect extremely weak magnetic fields.
Quantum computers: YBCO-based Josephson junctions are being explored as potential building blocks for quantum computers.
Superconducting Digital Circuits
Superconducting digital circuits can operate at much higher speeds and with lower energy consumption than conventional silicon-based circuits. This makes them attractive for applications in high-performance computing and communication systems.
Challenges and Future Prospects
While the potential of YBCO in electronics is promising, there are still significant challenges to overcome. These include the need for complex cooling systems and the development of reliable manufacturing processes. However, with continued research and development, YBCO-based electronic devices could revolutionize the field of electronics.
Conclusion
YBCO offers the potential for a wide range of electronic applications, from microwave devices to quantum computers. By overcoming the challenges associated with its use, YBCO could play a crucial role in shaping the future of electronics.
Chapter 12: YBCO in Medicine
Magnetic Resonance Imaging (MRI)
One of the most significant applications of YBCO is in the field of medicine. YBCO-based magnets are used to generate the strong magnetic fields required for magnetic resonance imaging (MRI), a powerful diagnostic tool that provides detailed images of the human body.
Advantages of YBCO-Based MRI
Higher field strengths: YBCO magnets can generate higher magnetic field strengths compared to conventional magnets, leading to improved image quality and resolution.
Reduced operating costs: YBCO magnets require less energy to operate than conventional magnets, reducing costs and environmental impact.
Smaller and lighter designs: YBCO magnets can be made smaller and lighter than conventional magnets, making them more suitable for portable MRI machines.
Other Medical Applications
In addition to MRI, YBCO has the potential for other medical applications, such as:
Magnetic particle imaging (MPI): MPI is a new imaging technique that uses magnetic nanoparticles to visualize biological processes. YBCO-based magnets can be used to generate the necessary magnetic fields for MPI.
Magnetic drug targeting: YBCO-based magnets can be used to target drugs to specific areas of the body, potentially improving the effectiveness of treatments.
Challenges and Future Prospects
While YBCO offers significant advantages for medical applications, there are still challenges to overcome. These include the need for complex cooling systems and the potential for interactions between the magnetic fields and medical devices. However, with continued research and development, YBCO could play a crucial role in advancing medical imaging and treatment technologies.
Conclusion
YBCO-based magnets have the potential to revolutionize the field of medicine by providing improved imaging capabilities and enabling new diagnostic and therapeutic techniques. As research and development in this area continue, we can expect to see even greater benefits from the use of YBCO in medical applications.
Chapter 13: The Search for Even Higher Tc Superconductors
Beyond YBCO
While YBCO remains a significant milestone in the field of high-temperature superconductivity, researchers continue to search for materials with even higher critical temperatures. Such materials could expand the range of potential applications and provide new insights into the fundamental physics of superconductivity.
Recent Advancements
In recent years, several new materials have been discovered that exhibit superconductivity at temperatures higher than that of YBCO. These include iron-based superconductors and hydrogen-rich compounds.
Iron-based superconductors: These materials have been shown to exhibit superconductivity at temperatures as high as 55 Kelvin.
Hydrogen-rich compounds: Under extreme pressure, certain hydrogen-rich compounds have been found to exhibit superconductivity at temperatures above 200 Kelvin.
Challenges and Opportunities
The development of new high-temperature superconductors presents both challenges and opportunities. Challenges include the difficulty of synthesizing these materials under extreme conditions and the complexity of understanding their electronic properties. However, the potential rewards are significant, as these materials could lead to breakthroughs in a wide range of applications.
The Future of Superconductivity
The search for higher temperature superconductors is an ongoing endeavor. As researchers continue to explore new materials and techniques, it is possible that we will one day discover a room-temperature superconductor. Such a material would have a profound impact on society and technology, opening up new possibilities in areas such as energy transmission, transportation, and electronics.
Conclusion
While YBCO remains a landmark achievement in the field of superconductivity, the quest for even higher temperature materials continues. The discovery of new superconductors could lead to significant advances in both fundamental physics and practical applications.
Chapter 14: The Challenges of Commercializing YBCO
Cost and Scalability
One of the major challenges to commercializing YBCO is the high cost associated with its production and use. The complex synthesis process and the need for specialized equipment can drive up costs. Additionally, scaling up the production of YBCO for large-scale applications can be difficult.
Reliability and Durability
Ensuring the reliability and durability of YBCO-based devices is another challenge. The superconducting properties of YBCO can be sensitive to factors such as temperature fluctuations, magnetic fields, and mechanical stress. Developing materials and technologies that can withstand these conditions is essential for commercialization.
Cooling Requirements
The need for cryogenic cooling systems to maintain YBCO in its superconducting state is a significant barrier to widespread adoption. These systems can be expensive, bulky, and energy-intensive. Developing more efficient and cost-effective cooling technologies is essential for commercializing YBCO applications.
Competing Technologies
YBCO faces competition from other materials and technologies, such as conventional conductors and alternative superconductors. These competing options may have lower costs or offer different advantages, making it challenging for YBCO to gain market share.
Conclusion
While YBCO offers significant potential for a wide range of applications, the challenges associated with commercializing this material are substantial. Overcoming these challenges will require continued research, development, and investment in the field of high-temperature superconductivity.
Chapter 15: The Impact of YBCO on Society and Technology
A Paradigm Shift
The discovery of YBCO and other high-temperature superconductors has the potential to revolutionize society and technology. The ability to harness the power of superconductivity at higher temperatures could lead to significant advancements in energy efficiency, transportation, electronics, and healthcare.
Potential Societal and Economic Benefits
Energy efficiency: Superconducting power transmission lines can reduce energy losses, leading to significant cost savings and environmental benefits.
Transportation: Maglev trains powered by superconducting magnets offer the potential for high-speed, energy-efficient transportation, reducing travel times and improving accessibility.
Electronics: Superconducting electronic devices can operate at much higher speeds and with lower energy consumption than conventional devices, leading to advancements in computing, communication, and other fields.
Healthcare: YBCO-based magnets are used in MRI machines, providing essential diagnostic tools for healthcare professionals.
Ethical Implications
The development and widespread adoption of YBCO-based technologies raise important ethical considerations. These include:
Environmental impact: While YBCO can help reduce energy consumption and greenhouse gas emissions, the production and use of this material may have environmental implications, such as resource consumption and waste generation.
Economic inequality: The benefits of YBCO-based technologies may not be distributed equitably, leading to economic inequality.
Security concerns: The potential for malicious use of superconducting technologies, such as in weapons or surveillance, raises ethical concerns.
Conclusion
The discovery of YBCO has opened up new possibilities for technological advancements. While there are challenges to overcome, the potential benefits of this material are significant. By carefully considering the ethical implications of YBCO-based technologies, we can ensure that they are developed and used in a responsible and sustainable manner.
Chapter 16: The Environmental Impact of YBCO
Resource Consumption and Waste Generation
The production and use of YBCO can have environmental implications. The mining and processing of the raw materials required for YBCO synthesis can lead to resource consumption and waste generation. Additionally, the production of cryogenic coolants needed to maintain YBCO in its superconducting state can also have environmental impacts.
Efforts to Reduce the Environmental Footprint
Researchers and engineers are working to reduce the environmental impact of YBCO. This includes efforts to develop more efficient synthesis methods, reduce waste generation, and explore alternative cooling technologies.
Sustainable materials: Researchers are investigating the use of more sustainable materials in the production of YBCO, such as recycled materials or materials with a lower environmental footprint.
Improved manufacturing processes: Advances in manufacturing techniques can help to reduce waste and improve the efficiency of YBCO production.
Alternative cooling technologies: Developing more energy-efficient and environmentally friendly cooling systems can help to reduce the environmental impact of YBCO applications.
Conclusion
While the environmental impact of YBCO is a concern, it is important to note that the potential benefits of this material in terms of energy efficiency and reduced greenhouse gas emissions can outweigh the negative impacts. By adopting sustainable practices and investing in research and development, it is possible to minimize the environmental footprint of YBCO while maximizing its benefits.
Chapter 17: The Role of YBCO in Scientific Research
Understanding Fundamental Physics
YBCO has played a crucial role in advancing our understanding of fundamental physics. The discovery of high-temperature superconductivity has challenged traditional theories and spurred the development of new theoretical frameworks.
Pairing mechanism: The search for the underlying pairing mechanism in YBCO has led to significant advances in our understanding of strongly correlated electron systems.
Quantum criticality: YBCO has been used to study quantum critical phenomena, where materials undergo phase transitions at absolute zero temperature.
Topological superconductivity: Recent studies have suggested that YBCO may exhibit topological superconductivity, a state of matter with exotic properties that could have applications in quantum computing.
Developing New Technologies
Beyond its applications in practical devices, YBCO has also been used as a platform for developing new technologies. For example, YBCO-based Josephson junctions have been used to create highly sensitive detectors for gravitational waves.
Conclusion
YBCO continues to be a valuable tool for scientific research. By studying its properties and behavior, scientists can gain insights into fundamental physics and develop new technologies with the potential to revolutionize various fields.
Chapter 18: The Role of Doping in YBCO
Tailoring Superconducting Properties
Doping, the introduction of foreign atoms into a material, can significantly alter its properties. In the case of YBCO, doping with various elements can be used to tune its superconducting transition temperature and other properties.
Rare Earth Doping
One of the most common types of doping in YBCO involves the substitution of rare earth elements for yttrium. Rare earth elements such as lanthanum, neodymium, and gadolinium can be added to YBCO to modify its superconducting properties.
Critical temperature: Doping with rare earth elements can increase or decrease the critical temperature of YBCO.
Carrier concentration: Doping can alter the concentration of charge carriers in YBCO, which can affect its superconducting properties.
Structural stability: Doping can also influence the structural stability of YBCO, which can impact its performance and durability.
Other Doping Elements
In addition to rare earth elements, YBCO can also be doped with other elements, such as strontium and calcium. These dopants can have different effects on the superconducting properties of YBCO.
Conclusion
Doping is a powerful tool for tailoring the properties of YBCO. By carefully selecting the dopant and the doping concentration, researchers can optimize YBCO for specific applications. Understanding the effects of doping on YBCO is essential for the development of new and improved superconducting materials.
Chapter 19: The Anisotropy of YBCO
Anisotropy in Crystal Structure
YBCO has an anisotropic crystal structure, meaning that its properties can vary depending on the direction in which they are measured. This anisotropy is due to the layered structure of YBCO, with Cu-O planes separated by insulating layers.
Anisotropy in Superconducting Properties
The superconducting properties of YBCO are also anisotropic. The critical current density, which is a measure of the maximum current that YBCO can carry without losing its superconducting state, is significantly higher in the Cu-O planes than in the direction perpendicular to these planes.
Impact of Anisotropy on Applications
The anisotropy of YBCO can have both positive and negative effects on its applications. On the one hand, the high critical current density in the Cu-O planes makes YBCO well-suited for applications that require high current-carrying capacity, such as power transmission and superconducting magnets. On the other hand, the anisotropy can make it challenging to design and fabricate YBCO-based devices that are isotropic in their properties.
Overcoming Anisotropy
Researchers have developed techniques to mitigate the effects of anisotropy in YBCO. These techniques include:
Grain orientation: By controlling the orientation of YBCO grains during synthesis, it is possible to produce materials with a more isotropic superconducting response.
Composite materials: Combining YBCO with other materials can help to reduce anisotropy and improve the overall performance of superconducting devices.
Conclusion
The anisotropy of YBCO is a key factor that must be considered in its design and applications. By understanding and addressing the effects of anisotropy, researchers can optimize the performance of YBCO-based devices and unlock their full potential.
Chapter 20: The Theory of High-Temperature Superconductivity
A Challenging Puzzle
Despite decades of research, the underlying mechanism responsible for high-temperature superconductivity in materials like YBCO remains a subject of intense debate. Numerous theories have been proposed, each with its own strengths and weaknesses.
Competing Theories
Some of the leading theories for high-temperature superconductivity include:
Magnetic fluctuation theory: This theory suggests that magnetic fluctuations in the Cu-O planes play a crucial role in pairing electrons.
Electronic correlation theory: This theory emphasizes the strong electronic interactions between copper and oxygen atoms in the Cu-O planes.
Excitonic mechanism: This theory proposes that excitons, bound electron-hole pairs, mediate the pairing of electrons.
D-wave pairing: This theory suggests that the Cooper pairs in high-temperature superconductors have a d-wave symmetry, which is different from the s-wave symmetry of conventional superconductors.
Challenges and the Road Ahead
Developing a unified theory of high-temperature superconductivity has proven to be a formidable challenge. The complexity of the materials involved and the interplay between various factors, such as electron-electron interactions, magnetic fluctuations, and lattice vibrations, have made it difficult to reach a consensus.
Despite these challenges, researchers continue to explore new theoretical approaches and experimental techniques to unravel the mysteries of high-temperature superconductivity. A definitive understanding of this phenomenon could have profound implications for both fundamental physics and technological applications.
Conclusion
The theory of high-temperature superconductivity remains a fascinating and challenging area of research. While a definitive understanding may still be elusive, the progress that has been made in recent years offers hope for future breakthroughs.
Chapter 21: The Future of YBCO and Superconductivity
Emerging Trends and Potential Breakthroughs
The field of superconductivity is constantly evolving, with new discoveries and advancements being made regularly. Some of the emerging trends and potential breakthroughs that may shape the future of YBCO and superconductivity include:
Higher temperature superconductors: Researchers continue to search for materials with even higher critical temperatures, which could expand the range of potential applications.
Improved materials and fabrication techniques: Advances in materials science and manufacturing processes could lead to more efficient and cost-effective production of YBCO and other superconductors.
New applications: As research progresses, new and innovative applications for YBCO and other superconductors may emerge, such as in quantum computing, energy storage, and medical devices.
Theoretical breakthroughs: A breakthrough in the theory of high-temperature superconductivity could provide new insights into the underlying mechanisms and pave the way for the development of even more advanced materials.
The Long-Term Outlook
The long-term outlook for YBCO and superconductivity is promising. As research and development continue, we can expect to see significant advancements in both fundamental understanding and practical applications. Superconductivity has the potential to revolutionize a wide range of industries and improve our quality of life.
Chapter 22: Levitation Technology
22.1 Introduction to Magnetic Levitation
Magnetic levitation (maglev) is a technology that uses magnetic fields to suspend objects. When a superconductor is cooled below its critical temperature, it becomes a perfect diamagnet, repelling magnetic fields. This property can be exploited to create levitation effects.
22.2 Levitation Using YBCO
Meissner Effect: When YBCO is cooled below its critical temperature (93 K), it expels magnetic fields from its interior, a phenomenon known as the Meissner effect. This creates a repulsive force between the YBCO and a permanent magnet or electromagnet.
Levitation Configurations: Various configurations can be used for levitation, such as the Halbach array, a permanent magnet arrangement that creates a strong, unidirectional magnetic field.
22.3 Applications of Levitation Technology
Transportation: Maglev trains are a promising application of levitation technology. They can achieve high speeds with low energy consumption and minimal friction.
Energy Storage: Flywheels made of superconducting materials can store energy efficiently. The levitated flywheel reduces friction, increasing its efficiency and lifespan.
Industrial Processes: Levitation can be used in various industrial processes, such as materials handling, manufacturing, and scientific research.
22.4 Challenges and Future Directions
Stability: Ensuring the stability of levitated objects is a critical challenge. Active control systems are often used to maintain the levitation height and prevent oscillations.
Cost: The cost of YBCO and the necessary cooling infrastructure can be a barrier to widespread adoption of levitation technology.
Efficiency: The efficiency of levitation systems depends on factors such as the strength of the magnetic fields, the cooling requirements, and the overall design.
Future Developments: Research is ongoing to develop new materials and techniques to improve the performance and reduce the cost of levitation technology.
Figure 22.1: A maglev train levitating above a track using superconducting magnets.
Figure 22.2: A levitated flywheel for energy storage.
Chapter 23: Aviation and YBCO
23.1 Introduction to Aviation and YBCO
The aviation industry is constantly seeking innovative technologies to improve efficiency, reduce emissions, and enhance safety. YBCO, with its unique properties, offers potential benefits for various aviation components.
23.2 Potential Applications of YBCO in Aviation
Electric Motors: YBCO can be used to create highly efficient electric motors for propulsion. These motors can operate at higher power densities and with lower losses compared to conventional motors.
Generators: YBCO can be used in generators to improve their efficiency and reduce weight. This can lead to increased fuel efficiency and reduced emissions.
Sensors: YBCO-based sensors can be used for a variety of applications in aviation, including detecting defects in materials, monitoring engine performance, and improving navigation systems.
Magnetic Bearings: YBCO can be used to create magnetic bearings for rotating machinery, such as generators and pumps. These bearings have no mechanical contact, reducing friction and wear.
23.3 Challenges and Future Directions
Weight and Cooling: Integrating YBCO into aircraft requires careful consideration of weight and cooling requirements. The weight of the superconducting material and the necessary cooling systems must be balanced against the potential benefits.
Reliability and Safety: Ensuring the reliability and safety of YBCO-based components in the harsh environment of aviation is a critical challenge.
Cost: The cost of YBCO and the associated infrastructure can be a barrier to widespread adoption in the aviation industry.
Future Developments: Research is ongoing to develop new YBCO-based components and improve their performance and reliability.
Figure 23.1: A conceptual illustration of an electric aircraft powered by YBCO-based motors.
Figure 23.2: A YBCO-based generator for aircraft applications.
23.4 Conclusion
YBCO offers promising potential for improving the efficiency, performance, and sustainability of the aviation industry. While there are challenges to overcome, continued research and development could lead to significant advancements in this field.
Chapter 24: Floating and YBCO
24.1 Introduction to Floating Structures
Floating structures have been used for centuries for various purposes, including transportation, storage, and habitation. YBCO, with its unique properties, offers potential benefits for creating innovative floating structures.
24.2 Levitation-Based Floating Structures
Magnetic Levitation: YBCO can be used to create levitated floating structures. By combining YBCO with magnets, it is possible to suspend objects above a liquid or gaseous medium.
Applications: Levitation-based floating structures could be used for water storage, transportation, or even space exploration.
24.3 Challenges and Future Directions
Stability: Ensuring the stability of levitated floating structures is a significant challenge. Factors such as waves, currents, and external forces can affect the stability.
Environmental Impact: The environmental impact of floating structures must be carefully considered. Factors such as pollution, habitat disruption, and climate change need to be addressed.
Cost: The cost of constructing and maintaining floating structures can be high, especially if they involve advanced technologies like YBCO.
Future Developments: Research is ongoing to develop new techniques and materials for creating more efficient and sustainable floating structures.
24.4 Other Floating Applications
Superconducting Cables: YBCO can be used to create superconducting cables that can be laid underwater or in other challenging environments. These cables can be used for power transmission or data communication.
Buoyancy Control: YBCO-based sensors can be used to monitor buoyancy and adjust the ballast of floating structures.
Figure 24.1: A conceptual illustration of a levitated floating platform using YBCO.
Figure 24.2: A superconducting cable for underwater power transmission.
24.5 Conclusion
YBCO offers promising potential for creating innovative floating structures. While there are challenges to overcome, continued research and development could lead to significant advancements in this field.
Chapter 25: Materials Similar to YBCO
25.1 Introduction to Related Superconductors
While YBCO has been a groundbreaking discovery in the field of high-temperature superconductivity, it is not the only material with such properties. Other superconductors, both cuprate and iron-based, have also shown promise for various applications.
25.2 Cuprate Superconductors
Bi-2212: Bismuth strontium calcium copper oxide (Bi-2212) is another important cuprate superconductor with a critical temperature of around 85 K. It has similar properties to YBCO but is often easier to fabricate and has a higher critical current density.
Tl-2223: Thallium barium calcium copper oxide (Tl-2223) has the highest critical temperature among the cuprate superconductors, reaching over 125 K. However, it is more difficult to synthesize and is less stable than YBCO and Bi-2212.
Other Cuprates: There are many other cuprate superconductors with varying properties and applications. These include La-214, Nd-214, and Hg-1223.
25.3 Iron-Based Superconductors
LaFeAsO: Lanthanum iron arsenic oxide was one of the first iron-based superconductors discovered. It has a critical temperature of around 26 K.
BaFeAs: Barium iron arsenic is another important iron-based superconductor with a critical temperature of around 38 K.
Other Iron-Based Superconductors: There are many other iron-based superconductors, such as FeSe and LiFeAs, each with its own unique properties and potential applications.
25.4 Comparison of YBCO and Related Superconductors
Property | YBCO | Bi-2212 | Tl-2223 | LaFeAsO | BaFeAs |
Critical Temperature (K) | 93 | 85 | 125 | 26 | 38 |
Crystal Structure | Tetragonal | Orthorhombic | Tetragonal | Tetragonal | Tetragonal |
Critical Current Density | High | High | Very high | Moderate | Moderate |
Fabrication | Moderate | Easier | Difficult | Moderate | Moderate |
Export to Sheets
25.5 Applications of Related Superconductors
Power Transmission: Both cuprate and iron-based superconductors can be used for power transmission cables, offering higher efficiency and lower energy losses compared to conventional conductors.
Magnetic Resonance Imaging (MRI): High-temperature superconductors can be used to create powerful magnets for MRI machines.
Electronics: Superconducting electronic devices can offer faster switching speeds and lower energy consumption compared to conventional devices.
25.6 Future Directions
Research continues to explore new superconductors and their potential applications. The discovery of materials with even higher critical temperatures and improved properties could revolutionize various industries.
Chapter 26: The Quest for Higher Temperature Superconductors
26.1 The Pursuit of Higher Tc
The discovery of YBCO marked a significant breakthrough in the field of superconductivity, but scientists continue to search for materials with even higher critical temperatures (Tc). A higher Tc would expand the range of potential applications and reduce the need for costly cooling systems.
26.2 Theoretical Approaches
BCS Theory: The Bardeen-Cooper-Schrieffer (BCS) theory, while successful for conventional superconductors, has limitations in explaining high-temperature superconductivity.
Alternative Theories: Various alternative theories have been proposed, including the resonating valence bond theory, the stripe theory, and the spin fluctuation theory. These theories attempt to explain the mechanism of high-temperature superconductivity.
26.3 Experimental Techniques
Material Synthesis: New materials are constantly being synthesized and tested for superconducting properties. Techniques such as chemical vapor deposition, molecular beam epitaxy, and flux growth are used to create these materials.
High-Pressure Experiments: Applying high pressure can alter the properties of materials, including their superconducting behavior. This technique has led to the discovery of new superconductors with higher Tc.
Doping and Substitution: Doping and substituting elements in the crystal lattice can modify the electronic properties of materials, sometimes leading to enhanced superconductivity.
26.4 Challenges and Future Directions
Complexity: High-temperature superconductors are often complex materials with intricate crystal structures. Understanding their properties and mechanisms requires sophisticated theoretical and experimental techniques.
Material Stability: Many new materials discovered with high Tc are often unstable or difficult to synthesize in large quantities.
Scalability: Scaling up the production of high-temperature superconductors for commercial applications remains a significant challenge.
Future Outlook: Despite these challenges, the pursuit of higher-temperature superconductors continues, with the hope of unlocking new applications and revolutionizing various industries.
Figure 26.1: A schematic diagram of a high-pressure apparatus used to study superconductivity under extreme conditions.
Figure 26.2: A periodic table highlighting the elements commonly found in high-temperature superconductors.
26.5 Conclusion
The quest for higher-temperature superconductors remains a fascinating and challenging endeavor. By combining theoretical approaches with experimental techniques, scientists hope to uncover new materials that can push the boundaries of superconductivity and revolutionize technology.
Chapter 27: Superconductivity in Extreme Conditions
27.1 High Pressure Superconductivity
Effect of Pressure: Applying high pressure can alter the crystal structure and electronic properties of materials, sometimes leading to enhanced superconductivity.
Discoveries: Several new superconductors have been discovered under high-pressure conditions, including hydrides with remarkably high critical temperatures.
Challenges: Creating and maintaining high-pressure conditions can be technically demanding, limiting the practical applications of these materials.
27.2 Low Temperature Phenomena
Quantum Hall Effect: At extremely low temperatures and in strong magnetic fields, two-dimensional electron gases can exhibit the quantum Hall effect, where the electrical conductivity becomes quantized.
Fractional Quantum Hall Effect: A more exotic phenomenon, the fractional quantum Hall effect, occurs when the filling factor of the electron gas is a rational fraction. This effect is related to the formation of quasiparticles known as anyons.
Superconducting States: Superconductivity itself can exhibit interesting phenomena at low temperatures, such as the Josephson effect and the Little-Parks effect.
27.3 Extreme Conditions and Future Directions
Exploring the Limits: Scientists continue to explore the limits of superconductivity under extreme conditions, such as ultra-high pressures or ultra-low temperatures.
Potential Applications: Understanding the behavior of superconductors in extreme conditions could lead to new applications, such as quantum computing and energy storage.
Figure 27.1: A schematic diagram of a high-pressure cell used for studying superconductivity.
Figure 27.2: The quantum Hall effect, where the electrical conductivity becomes quantized in a two-dimensional electron gas.
27.4 Conclusion
Superconductivity in extreme conditions offers a rich field of study, with potential applications in various areas of science and technology. By understanding the behavior of superconductors under these conditions, scientists can gain valuable insights into the fundamental properties of matter and explore new frontiers in materials science.
Chapter 28: Superconducting Quantum Computing
28.1 Introduction to Quantum Computing
Quantum computing is a revolutionary field that leverages the principles of quantum mechanics to perform calculations that would be impractical or impossible on classical computers. Superconducting materials have emerged as a promising platform for building quantum bits (qubits), the fundamental units of quantum information.
28.2 Superconducting Qubits
Josephson Junctions: Josephson junctions, formed by sandwiching a thin insulating barrier between two superconducting materials, are essential components of superconducting qubits.
Qubit Types: Different types of superconducting qubits have been developed, including transmons, flux qubits, and phase qubits. Each type has its own advantages and disadvantages in terms of coherence time, controllability, and scalability.
28.3 Quantum Algorithms and Applications
Quantum Chemistry: Quantum computers can simulate chemical reactions with unprecedented accuracy, potentially leading to breakthroughs in drug discovery and materials science.
Machine Learning: Quantum machine learning algorithms have the potential to solve complex optimization problems and accelerate the development of artificial intelligence.
Cryptography: Quantum computers could break certain classical cryptographic algorithms, necessitating the development of quantum-resistant encryption methods.
28.4 Challenges and Future Directions
Coherence: Maintaining the quantum state of qubits for a sufficiently long time is a major challenge. Efforts are underway to improve coherence times through various techniques.
Scalability: Building large-scale quantum computers requires scaling up the number of qubits while maintaining their coherence and control.
Error Correction: Quantum error correction techniques are essential for mitigating the effects of noise and errors in quantum circuits.
Quantum Supremacy: Demonstrating quantum supremacy, where a quantum computer can perform a task that is beyond the capabilities of classical computers, is a major milestone in the field.
Figure 28.1: A schematic diagram of a superconducting qubit based on a Josephson junction.
Figure 28.2: A quantum circuit composed of superconducting qubits.
28.5 Conclusion
Superconducting quantum computing is a rapidly evolving field with the potential to revolutionize various industries. By addressing the challenges of coherence, scalability, and error correction, researchers hope to realize the full potential of this transformative technology.
Chapter 29: Energy Storage and YBCO
29.1 Superconducting Magnetic Energy Storage (SMES)
Principles: SMES systems store energy in the form of a magnetic field generated by a superconducting coil. YBCO, with its high critical current density, is an ideal material for these coils.
Advantages: SMES systems offer high energy density, rapid charge/discharge rates, and long cycle life. They are particularly well-suited for grid stabilization and peak load shaving.
Challenges: The cost of constructing and operating SMES systems can be high, and there are challenges related to cooling and cryogenics.
29.2 Applications of SMES
Grid Stabilization: SMES systems can be used to balance supply and demand on the electrical grid, preventing blackouts and brownouts.
Peak Load Shaving: By storing energy during off-peak hours and releasing it during peak demand periods, SMES systems can reduce the overall load on the grid.
Renewable Energy Integration: SMES systems can help integrate renewable energy sources, such as wind and solar, into the grid by storing excess energy during periods of high generation.
29.3 YBCO-Based SMES Systems
Coil Design: The design of the superconducting coil is critical for the performance of a SMES system. YBCO can be used to create coils with high current densities and low losses.
Cooling Systems: Efficient cooling systems are essential to maintain YBCO in its superconducting state. Cryogenic systems are used to cool the coils to the required temperature.
Integration with the Grid: SMES systems must be integrated with the electrical grid to be effective. Control systems are used to manage the charging and discharging of the system.
Figure 29.1: A schematic diagram of a superconducting magnetic energy storage system.
29.4 Future Directions
Cost Reduction: Efforts are underway to reduce the cost of constructing and operating SMES systems, making them more competitive with other energy storage technologies.
Increased Capacity: Developing larger-scale SMES systems can increase their potential for grid stabilization and renewable energy integration.
Hybrid Systems: Combining SMES with other energy storage technologies, such as batteries, can create more flexible and efficient energy storage solutions.
29.5 Conclusion
YBCO-based SMES systems offer a promising solution for energy storage and grid stabilization. As research and development continue, these systems are expected to play an increasingly important role in the transition to a sustainable energy future.
Chapter 30: The Future of YBCO and Superconductivity
30.1 Technological Roadmap
Advancements in YBCO: Continued research and development aim to improve the properties of YBCO, such as critical current density and grain size, to enhance its performance in various applications.
New Materials: The search for new superconductors with higher critical temperatures and improved properties remains an active area of research.
Integration with Existing Technologies: Developing strategies to integrate YBCO and other superconductors into existing technological infrastructures will be crucial for their widespread adoption.
30.2 Societal and Economic Impacts
Energy Efficiency: The widespread adoption of superconductivity technology could lead to significant energy savings in sectors such as transportation, power transmission, and electronics.
Economic Growth: The development and commercialization of superconductivity applications could create new industries and job opportunities.
Environmental Benefits: Superconductivity can contribute to a more sustainable future by reducing energy consumption and greenhouse gas emissions.
30.3 Challenges and Opportunities
Cost: The cost of producing and implementing superconductivity technology remains a significant barrier to widespread adoption.
Infrastructure: Developing the necessary infrastructure to support superconductivity applications, such as cooling systems and power grids, will be essential.
Public Awareness: Increasing public awareness and understanding of superconductivity will be crucial for fostering its acceptance and adoption.
30.4 Conclusion
The future of YBCO and superconductivity is bright, with the potential to revolutionize various aspects of society and the economy. By addressing the challenges and capitalizing on the opportunities, we can harness the power of superconductivity to create a more sustainable and prosperous future.
Chapter 31: Ethical Considerations and Sustainability
31.1 Environmental Impact
Resource Consumption: The production of YBCO and other superconductors can require significant resources, including rare earth elements and energy.
Waste Generation: The manufacturing process can generate waste products, including chemicals and hazardous materials.
Cooling Systems: The operation of superconductors often requires the use of cryogenic cooling systems, which can have environmental implications due to energy consumption and refrigerant emissions.
31.2 Social and Economic Implications
Job Creation: The development and commercialization of superconductivity technology can create new industries and jobs, particularly in research, development, manufacturing, and applications.
Economic Disruption: The introduction of new technologies can disrupt existing industries and lead to job losses in some sectors.
Social Equity: The benefits of superconductivity technology may not be distributed equitably, with potential disparities between developed and developing countries.
31.3 Ethical Considerations
Military Applications: The potential for military applications of superconductivity technology raises ethical concerns, particularly regarding weapons and surveillance.
Privacy and Security: The development of quantum computers based on superconducting materials could have implications for privacy and security, as they could potentially break existing encryption methods.
31.4 Sustainability and the Future
Sustainable Development: The development and use of superconductivity technology should be aligned with sustainable development goals, including reducing environmental impact, promoting social equity, and ensuring economic prosperity.
Ethical Frameworks: Establishing ethical frameworks and guidelines for the development and use of superconductivity technology can help address potential concerns and ensure responsible innovation.
Figure 31.1: A diagram illustrating the potential environmental impacts of superconductivity technology.
31.5 Conclusion
The development and use of superconductivity technology present both opportunities and challenges. By carefully considering the ethical, social, and environmental implications, we can ensure that superconductivity contributes to a more sustainable and equitable future.
Subscribe to my newsletter
Read articles from Nik Shah xAI directly inside your inbox. Subscribe to the newsletter, and don't miss out.
Written by

Nik Shah xAI
Nik Shah xAI
Nikhil Pankaj Shah, CFA CAIA, is a visionary LLM GPT developer, author, and publisher renowned for his work with xAi Robotics and Cohere Capital. He holds a background in Biochemistry from Harvard University and advanced degrees in Finance & Accounting from Northeastern University, having initially studied sports management at UMass Amherst. Nik Shah xAi is a dedicated advocate for sustainability and ethics, he is known for his work in AI ethics, neuroscience, psychology, healthcare, athletic development, and nutrition-mindedness. Nikhil Shah explores profound topics such as primordial soul consciousness, autonomous mobility, and humanoid robotics, emphasizing innovative technology and human-centered principles to foster a positive global impact. AUTHORITATIVE WORK for nikshahxai Equity in Athletics | Advocating Gender Equity & Participation in Sports, Empowering Women (ISBN 979-8339961444) Mastering AI | From Fundamentals to Future Frontiers (ISBN 979-8338704448, 979-8338895238) Pure Intelligence | The Human Mind Unleashed (ISBN 979-8338450369) Zero Net Mastery | Balancing Caloric Intake with Precision (ISBN 979-8338452974) Paramatman | The Primordial Self: Embracing the King of the Universe, Soul Consciousness, and Holistic Existence (ISBN 979-8339898887) Mastering Medical Healthcare (ISBN 979-8338685747) Psychology Mastered (ISBN 979-8338894644, 979-8338680728) Contributing Authors to all my publishings: Nanthaphon Yingyongsuk, Rushil Shah, Sean Shah, Sony Shah, Darshan Shah, Kranti Shah, Rajeev Chabria, John DeMinico, Gulab Mirchandani